The Power and the Promise of Peptide Therapeutics
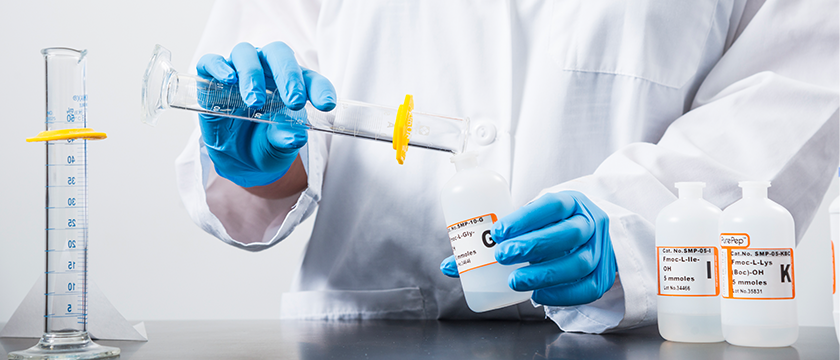
Thousands of peptides are produced in the body to perform a wide range of functions. This makes peptides a promising route toward well-tolerated and effective treatments. Therapeutic peptides are now being used to treat a range of conditions, including metabolic diseases, cancer, cardiovascular and infectious diseases, pain and hematological diseases. This has been the result of considerable efforts in R&D, including the development of advanced methods for solid-phase
peptide synthesis to prepare customized peptides with improved function, and greater resistance to degradation.
Peptides provide diversity in a dynamic pipeline
Therapeutic peptides have many advantages, including high activity, great chemical and biological diversity, and low toxicity. In addition, thanks to advances in solid-phase peptide synthesis that enable efficient synthesis with high purity, peptides are relatively easy to produce at low cost compared to protein-based biologicals. These advantages are driving a dynamic peptide drug discovery process, based on the efforts of hundreds of academic groups and the formation of peptide therapeutics companies.
In the last five years (2015–2019), the U.S. Food Drug Administration (FDA) has authorized 15 peptides or peptide-containing molecules of a total of 208 new drugs (150 new chemical entities and 58 biologics; 1). Peptide-based drug candidates include a range of structures, from small to large, including cyclic or branched constructs, conjugation to antibodies, or incorporation of radionuclides.
Three basic types of therapeutic peptide
Therapeutic peptides can be divided into native, analog, and heterologous peptides. Analog peptides are the majority of peptides in clinical trials and have adapted sequences to improve therapeutic potential. Heterologous peptides are not related to native sequences and are discovered by screening libraries of naturally produced compounds of other species, by rational and computational drug design, or by phage display. Such peptides may have longer half-lives and novel functions, but there is also the increased risk of side-effects.
A broad range of applications
Most naturally occurring peptides bind to cell surface receptors to trigger intracellular responses, and function as hormones, neurotransmitters, growth factors, ion channel ligands, and anti-infectives. Therapeutic peptides can function simply, in peptide replacement, such as insulin, or be used to expedite the delivery of cytotoxic substances or imaging agents into specific cells.
Therapeutic peptides can also function as antivirals, targeting HIV, influenza, or hepatitis, generally by inhibiting the replication cycle, and this ability has come into particular focus in the intensive search for therapeutic solutions during the ongoing COVID-19 pandemic. The SARS-CoV-2 virus invades host cells by binding the human angiotensin-converting enzyme 2 (ACE2) receptor on the cell surface through its viral spike protein. The interfaces involved in these protein-protein interactions (PPIs) are dynamic and often planar, which puts special demands on the design of drugs that can ‘stick’ to the flat surfaces of the druggable target protein. The most promising PPI disruptors are peptides, which are better at disrupting the broad surface interactions involved than small-molecule inhibitors, and various groups are therefore looking into how peptides can be used to disrupt the SARS-CoV-2/ACE2 interaction (2).
Why are peptides used as therapeutics?
The advantages of peptides as therapeutics, and also as vaccines are many:
- Fully-defined composition
- Large-scale production is affordable
- Water-soluble, stable in storage, can be freeze-dried
- No biological contamination
- Minimal allergic and autoimmune response
- Can be customized as multipurpose therapeutic
Examples of therapeutic peptides
Spider venom peptides for non-opioid pain relief
Pain research took a major step forward when it was discovered that a voltage-gated sodium channel (NaV1.7) plays a major role in human pain perception, sparking the search for novel analgesics that could act by blocking NaV1.7. The challenge has been to identify inhibitors that are highly selective for NaV1.7, as only four of the nine voltage-gated NaV channel sub-types are involved in pain signaling while others have critical roles in heart and muscle function. The search for new analgesics has led to the discovery of peptides in spider venom that bind to voltage-gated ion channels involved in pain. A research group headed by Christina Schroeder and based at the University of Queensland, Australia has used SPPS to prepare variants of these gating modifier toxins (GMTs) on model membranes and investigate how individual peptides interact with the channels and surrounding membranes to achieve selective channel inhibition 3, 4.
Figure 1. The hydrophobic patch and surrounding cationic ring in spider-venom GMTs promotes NaV channel promiscuity. A representative GMT is shown with hydrophobic patch (green), positively charged residues (blue), and anionic amino acid residues (red). A detail from Figure 10, Agwa et al, 2018, reference 3.
Cyclotide scaffolds stabilize epitopes
The poor proteolytic stability of natural, linear peptides limits their therapeutic potential, and one way of increasing stability can be to exploit cyclotides, which are cyclic peptides that resist proteolysis due to their highly constrained structure. A research group based at the University of Queensland, Australia, and also including Christina Schroeder, has shown that non-immunogenic cyclotides linked to VHH7, a nanobody that targets murine class II MHC molecules, can be a simple approach to targeting antigen-presenting cells (APCs5). Cyclotides may therefore be valuable scaffolds for the construction of epitopes that need to be presented in a constrained form that is also resistant to proteolysis.
Figure 2. Cyclotides can be valuable scaffolds that enable epitopes to be presented in a constrained form that is resistant to proteolysis. Figure 1a, Kwon et al, 2018, reference 5.
Epigenetic approaches to treat blood cancer
Epigenetic changes are thought to be involved in many, perhaps all cancers, which makes methylation modulators promising drug candidates. Since the first peptide-derived epigenetic drug (romidepsin) was approved for the treatment of different forms of T cell lymphoma in 2009 and 2011, research has continued at an ever-increasing pace to find new ways to modulate epigenetics in cancer therapy. For example, a research team headed by Hao Jiang, based at the Department of Biochemistry and Molecular Genetics, University of Alabama, USA, has focused on the chromatin modulator DPY30, which facilitates histone H3K4 methylation by directly binding to ASH2L and plays an important role in hematologic malignancies6. The team used a peptide to disrupt the binding of DPY30 to ASH2L in order to investigate the domain on DPY30 that regulates cancer growth. Their results demonstrate the potential for targeting DPY30/ASHL2 binding to treat hematologic malignancy, and the next step could be the development of even more effective molecules to target DPY30, based on peptidomimetics and small molecule inhibitors with better pharmacological properties.
The need to synthesize complex peptides
While peptides have many advantages as therapeutics, the examples above illustrate the need to ensure that peptides are resistant to degradation and can maintain their structure. Achieving this often requires modifications that involve the synthesis of so-called ‘complex peptides’.
As we noted in a previous blog post on peptide-based vaccines , peptides can be complex in many ways:
- Longer peptides, >30 amino acids, are a challenge to synthesize, for example chemokines or histones.
- Highly hydrophobic peptides such as beta-amyloids involved in Alzheimer’s disease and extensively used in research.
- Cyclic peptides, in efforts to improve the rigidity, stability and resistance to degradation of therapeutic peptides. These are stapled, disulfide-bridged, bicyclic, or cyclized head-to-tail. Cyclotides, for example, are stable to proteolytic attack and have high thermal stability due to their highly constrained structure created by a head-to-tail cyclic backbone and three disulfide bonds that form a cystine-knotted core.
- Peptides can be branched, include side-chain modifications, phospho-peptides or be cysteine rich.
- Post-translational modifications (PTMs) of native peptides may need to be mimicked to achieve maximum effect.
- The need to maintain stereochemistry (risk of racemization during synthesis).
Meeting these challenges means using a peptide synthesizer that can handle complex chemistries while minimizing cross-contamination, dead volumes, and reagent carryover. This is especially important for the synthesis of long sequences, in which even small amounts of impurities, side products, and incomplete reactions over many cycles can drastically reduce the final purity and yield of desired peptides. Aggregation, secondary structure, steric hindrance, and conformational effects can still pose challenges in synthesis, and real-time monitoring optimizes reaction times to ensure complete deprotection.
Conclusions
We have come a long way since insulin was first extracted a hundred years ago and later used in replacement therapy to treat diabetes. The many advantages of peptides as therapeutics are now being realized through solid-phase peptide synthesis that can generate complex molecules with fine-tuned functionality and resistance to degradation. You can find out more about how the synthesis challenges posed by complex peptides can be met by downloading the White Paper ‘Meeting the challenge of synthesizing complex therapeutic peptides’.
References:
1 Editorial - Peptide Therapeutics 2.0. de la Torre, BG and Albericio, F. Molecules 2020, 25, 2293; doi:10.3390/molecules25102293
2 Evidence supporting the use of peptides and peptidomimetics as potential SARS-CoV-2 (COVID-19) therapeutics. VanPatten, S et al. Future medicinal chemistry. July 2020 DOI: 10.4155/fmc-2020-0180
3 Gating modifier toxins isolated from spider venom: Modulation of voltage-gated sodium channels and the role of lipid membranes. Agwa AJ et al, J Biol Chem. 2018 Jun 8;293(23):9041-9052. doi: 10.1074/jbc.RA118.002553. Epub 2018 Apr 27.
4 Webinar: Venom peptides: Rethinking voltage-gated sodium channel inhibition, Christina Schroeder
5 Targeted delivery of cyclotides via conjugation to a nanobody. Kwon S et al. ACS Chem. Biol., 2018, 13 (10), 2973–2980. DOI: 10.1021/acschembio.8b00653
6 Specific inhibition of DPY30 activity by ASH2L-derived peptides suppresses blood cancer cell growthhttps://www.ncbi.nlm.nih.gov/pubmed/31251903. Shah, KK et al. Experimental Cell Research. Vol 382, Issue 2, 15 September 2019, 111485.